Malonic Ester Synthesis
In this tutorial, I want to talk about the Malonic Ester Synthesis, which is actually a bit of a misnomer. The thing is, we are not synthesizing the malonic ester itself; rather, the malonic ester serves as our starting material. By the end of this synthesis, we will obtain a carboxylic acid with different groups attached to it. The Malonic Ester Synthesis is actually part of a family of synthetic transformations, with the two most common variations being the Malonic Ester Synthesis itself and the Acetoacetic Ester Synthesis.
General Scheme of the Malonic Ester Synthesis
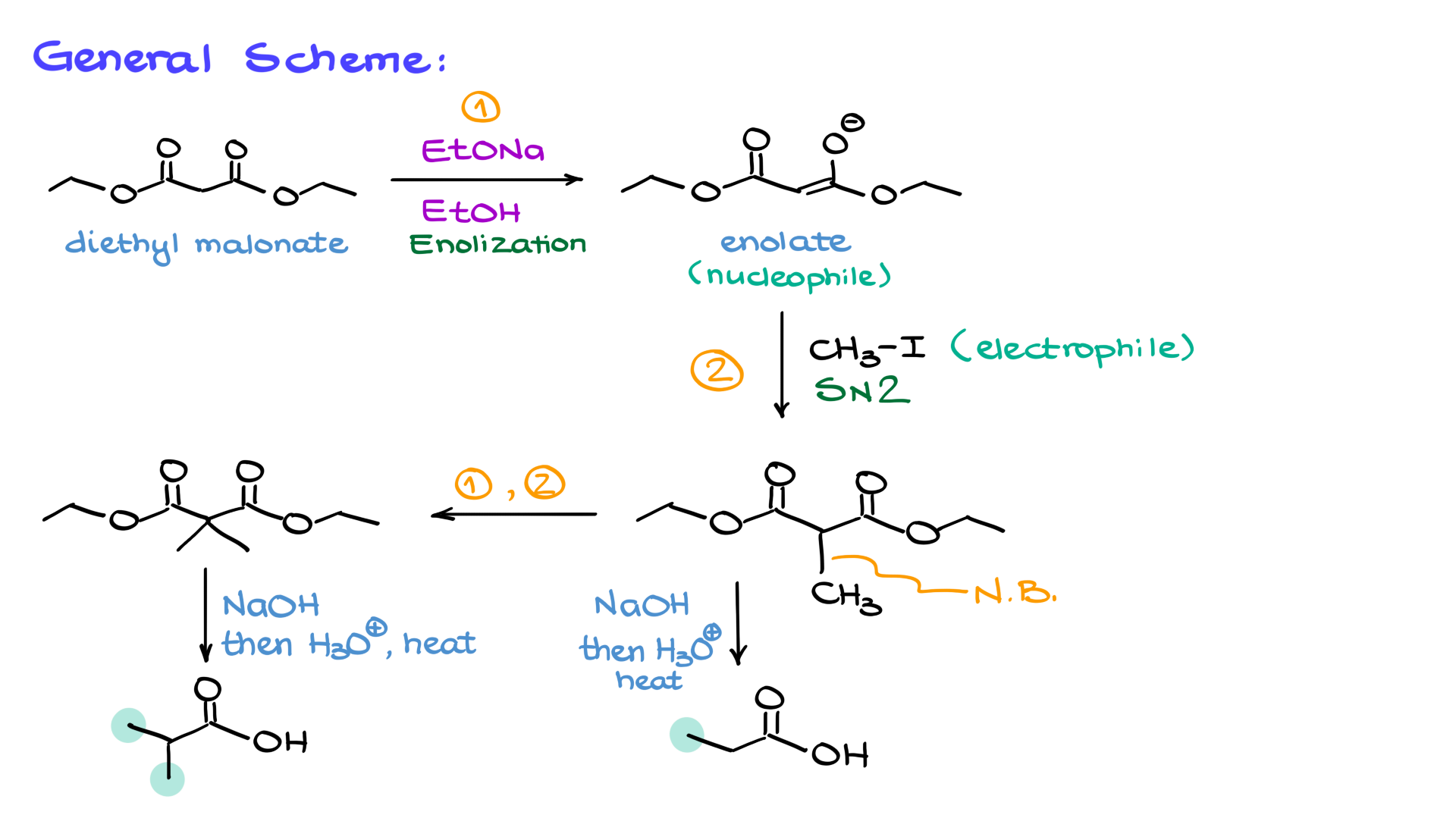
Let’s start by looking at the general scheme of the Malonic Ester Synthesis. As I mentioned earlier, we begin with malonic ester, specifically diethyl malonate in this case. The first step in the synthesis is enolization, where we deprotonate the malonic ester to form the corresponding enolate. Since the enolate is a nucleophile, it can then react with an electrophile—typically an alkyl halide. This reaction forms a new carbon-carbon bond between the carbon positioned between the two carbonyl groups and the carbon introduced by the electrophile.
At this point, we have two possible pathways. We can either:
- Repeat the enolization and alkylation cycle, introducing a second alkyl group before proceeding to hydrolysis and decarboxylation, which will yield a carboxylic acid with two additional groups.
- Proceed directly to hydrolysis and decarboxylation after adding just one alkyl group, resulting in a carboxylic acid with a single new substituent.
The choice of pathway depends on the structure of the target molecule. Since this synthesis involves multiple steps, let’s break each one down to ensure a clear understanding.
Step 1: Enolization
To begin, if we take malonic ester and treat it with a base such as ethoxide, we can easily remove the proton from the position between the two carbonyl groups, forming an enolate. This enolate is highly stabilized by resonance. While I won’t draw out the resonance structures here for the sake of time, you should practice sketching them yourself.
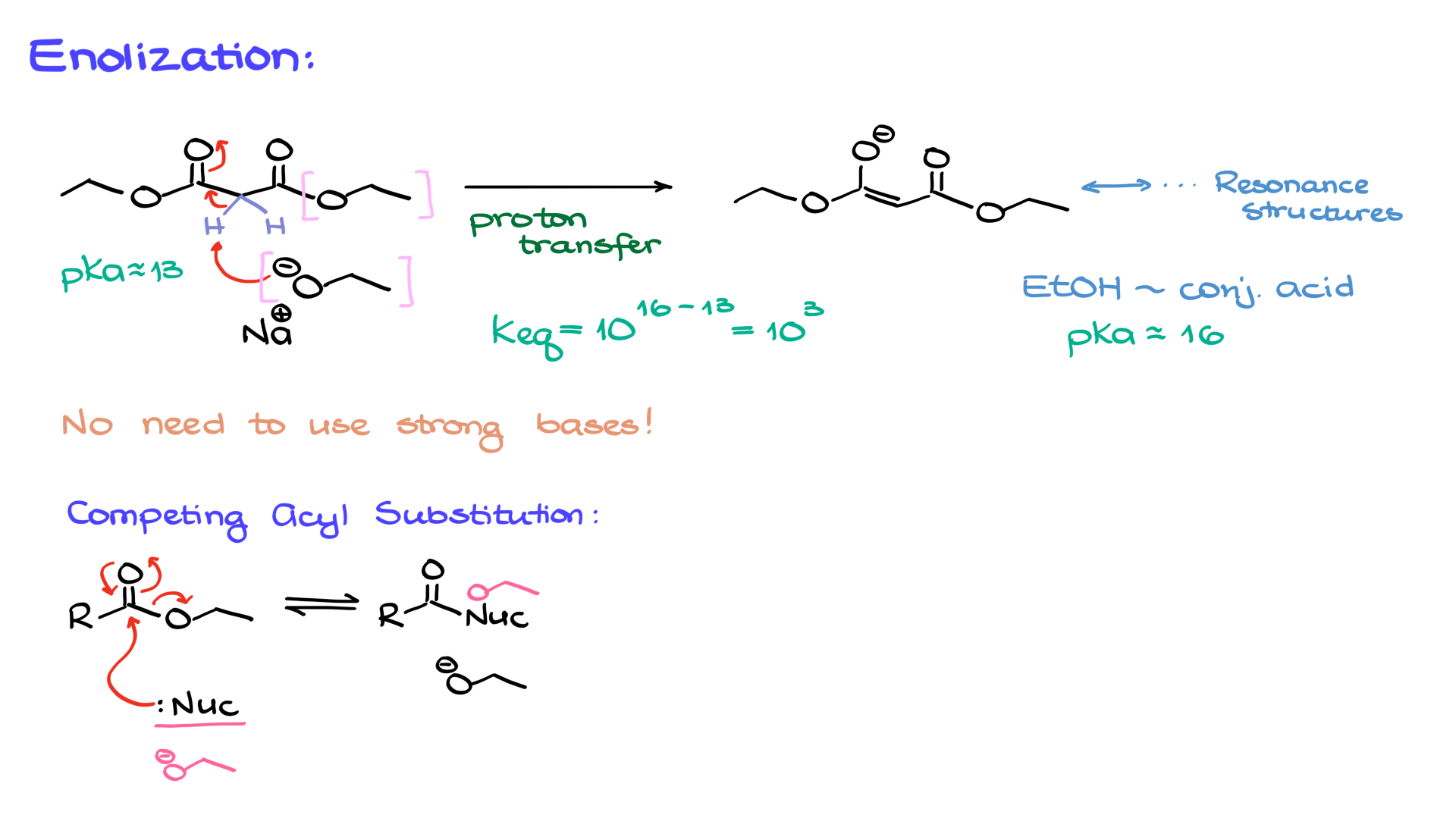
An important point to note is that this proton transfer is nearly quantitative. The pKa of malonic ester is about 13, while the conjugate acid of our base (ethanol) has a pKa of around 16. Using the equilibrium constant equation (10^(pKa of conjugate acid – pKa of acid)), we find that the equilibrium constant is approximately 10³—a fairly large value. This means that the reaction strongly favors enolate formation, and almost all of the starting material is converted into the enolate form.
However, there is a crucial detail to remember: the base used must match the ester group in the starting material. If we have an ethyl ester, we must use ethoxide as the base. This prevents competing side reactions such as transesterification or hydrolysis, which could otherwise introduce unwanted byproducts. If the nucleophile (the base) matches the ester group, the reaction simply regenerates the starting material, eliminating side reactions from consideration.
Step 2: Alkylation
Next, we take the enolate formed in the previous step and react it with an electrophile—typically an alkyl halide. This is a fairly typical alkylation of enolates step. Let’s use methyl iodide as an example. The nucleophilic enolate attacks the electrophilic alkyl halide, displacing the iodide and forming a new carbon-carbon bond.
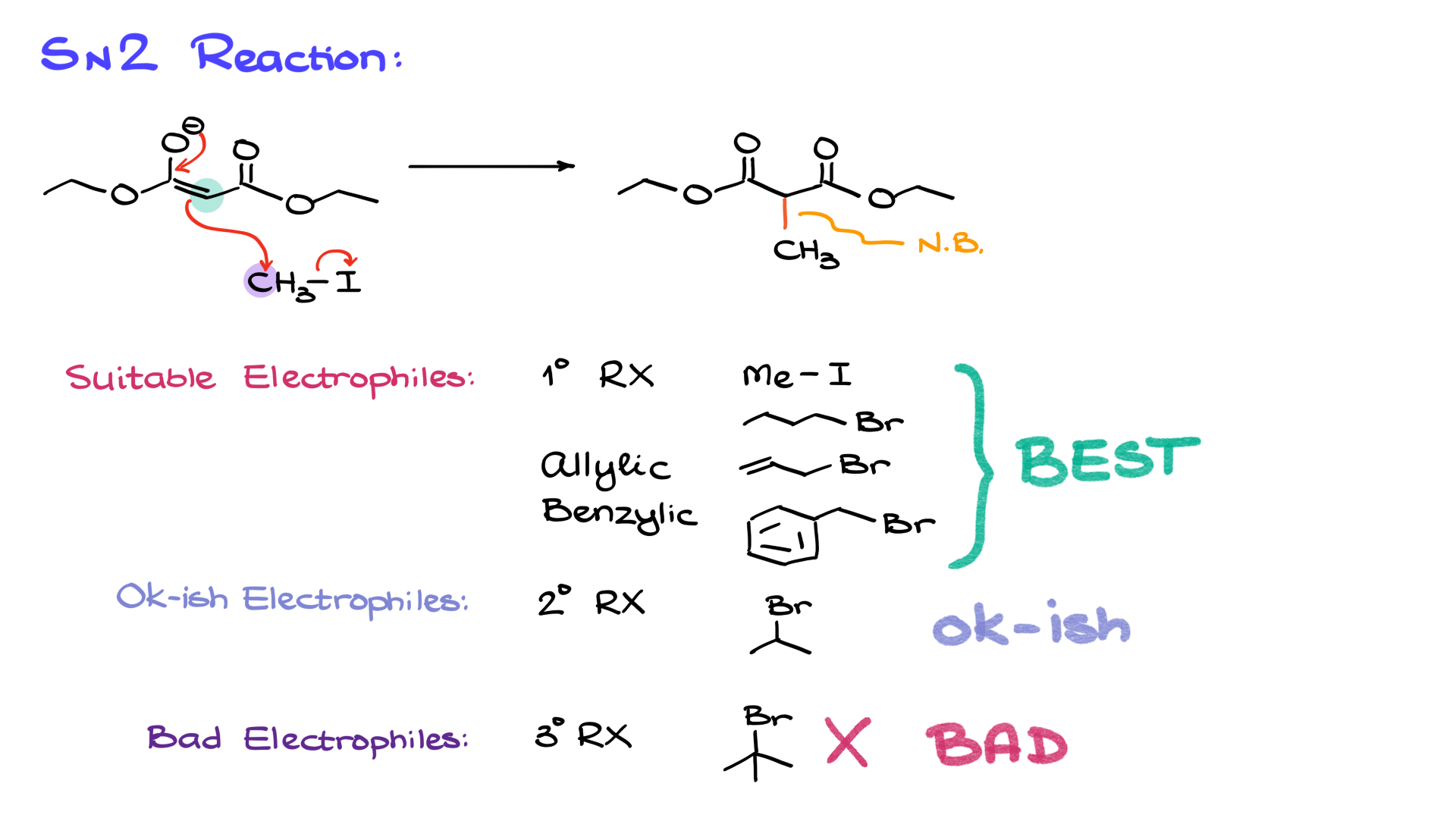
However, there are limitations to this reaction. Since it proceeds via an SN2 mechanism, steric hindrance plays a significant role in determining the feasibility of the reaction.
• Best electrophiles: Primary alkyl halides (e.g., methyl iodide, butyl bromide), allylic halides (e.g., allyl bromide), and benzylic halides (e.g., benzyl bromide).
• Acceptable but less ideal electrophiles: Smaller secondary alkyl halides (e.g., isopropyl bromide).
• Poor electrophiles: Tertiary alkyl halides (e.g., tert-butyl bromide), which are unsuitable due to steric hindrance preventing an SN2 reaction.
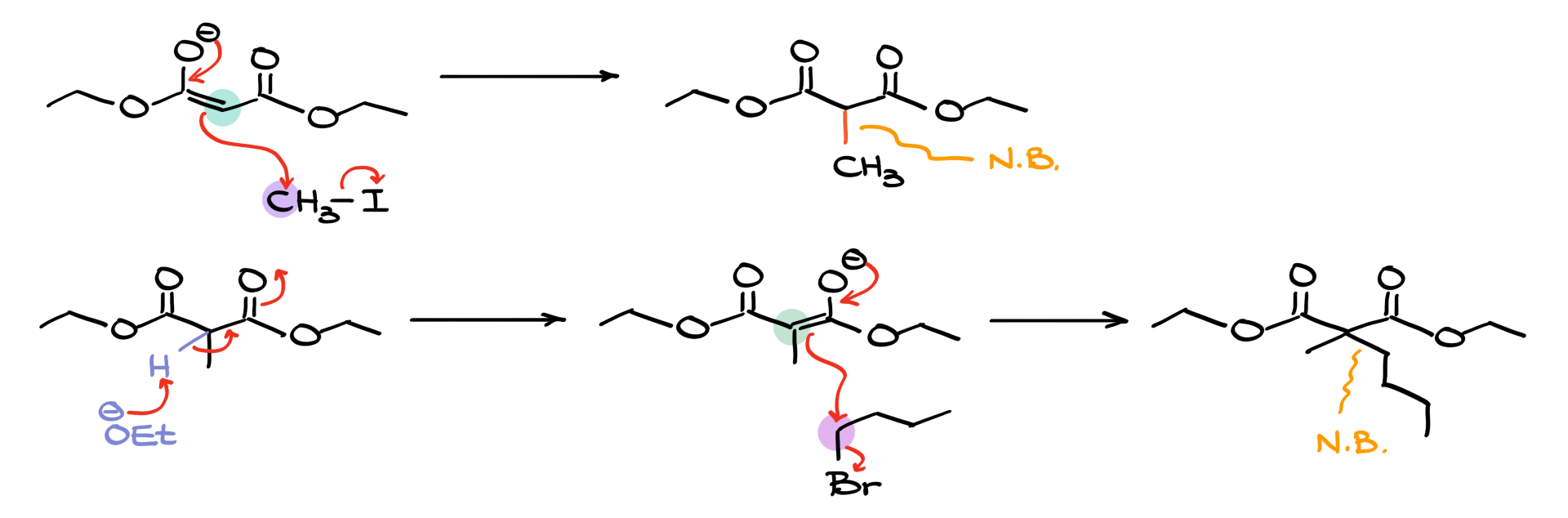
We can perform this alkylation step once or repeat the enolization-alkylation cycle to introduce a second alkyl group. If we repeat the process, we take the mono-alkylated product, enolize it again, and react it with a second alkyl halide (which can be the same or different from the first one). This allows for the formation of more complex molecules.
Step 3: Hydrolysis and Decarboxylation
Once the alkylation step is complete—whether we introduce one or two new groups—we move on to hydrolysis and decarboxylation. The goal here is to convert the ester groups into carboxylic acids and then remove one of them through decarboxylation.
There are two common methods for hydrolysis:
1. Acidic hydrolysis: Treating the ester with aqueous acid leads to protonation of the ester carbonyl, making it more electrophilic. Water then attacks, forming a tetrahedral intermediate, which eventually eliminates the alcohol group to give a carboxylic acid. If we have two esters, both will be hydrolyzed to dicarboxylic acid (malonic acid).
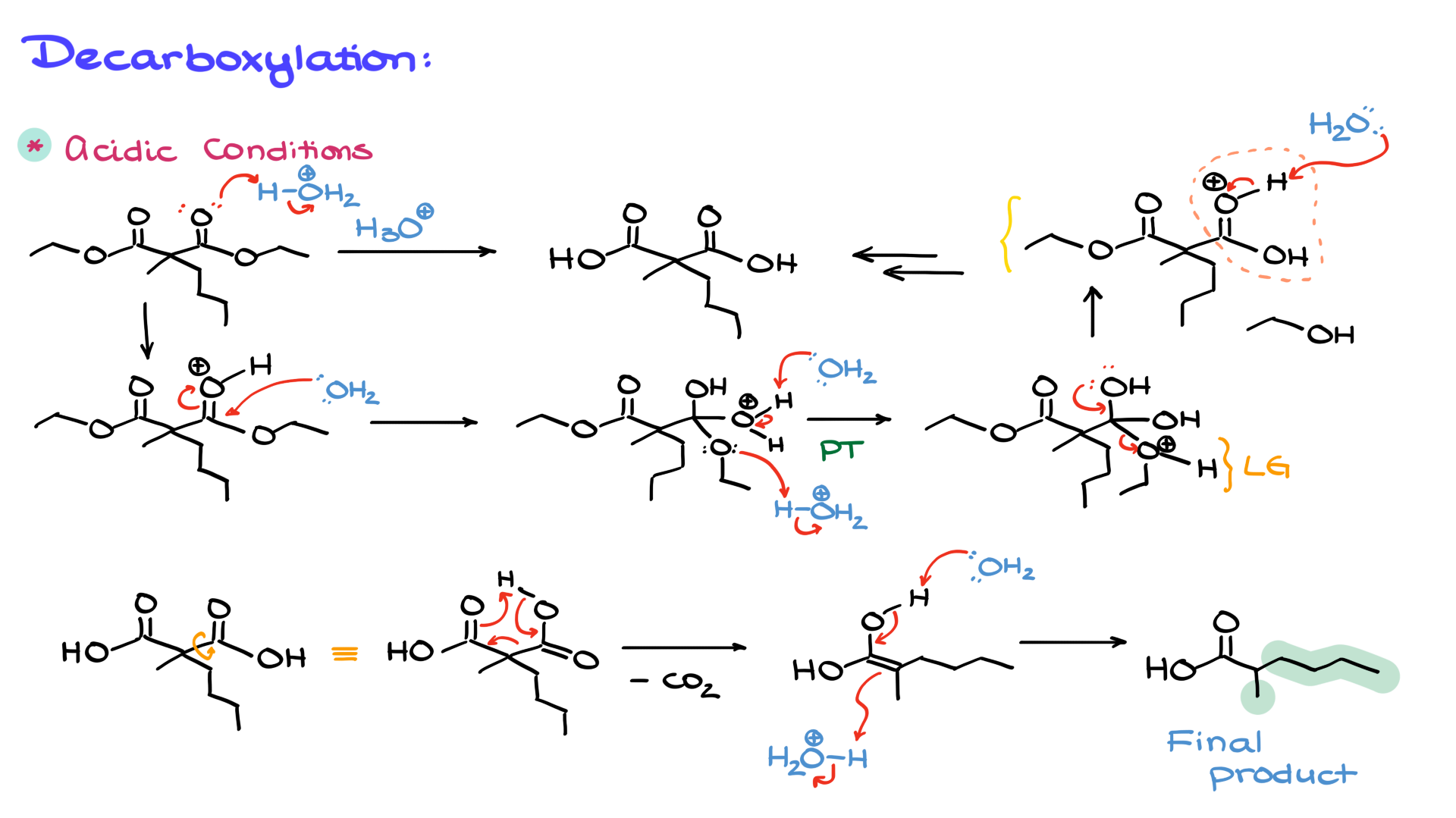
2. Basic hydrolysis (saponification): Using a strong base like hydroxide, we generate the carboxylate anion instead of the free carboxylic acid. After completing the hydrolysis, we switch to acidic conditions to protonate the carboxylate groups, yielding the corresponding dicarboxylic acid.
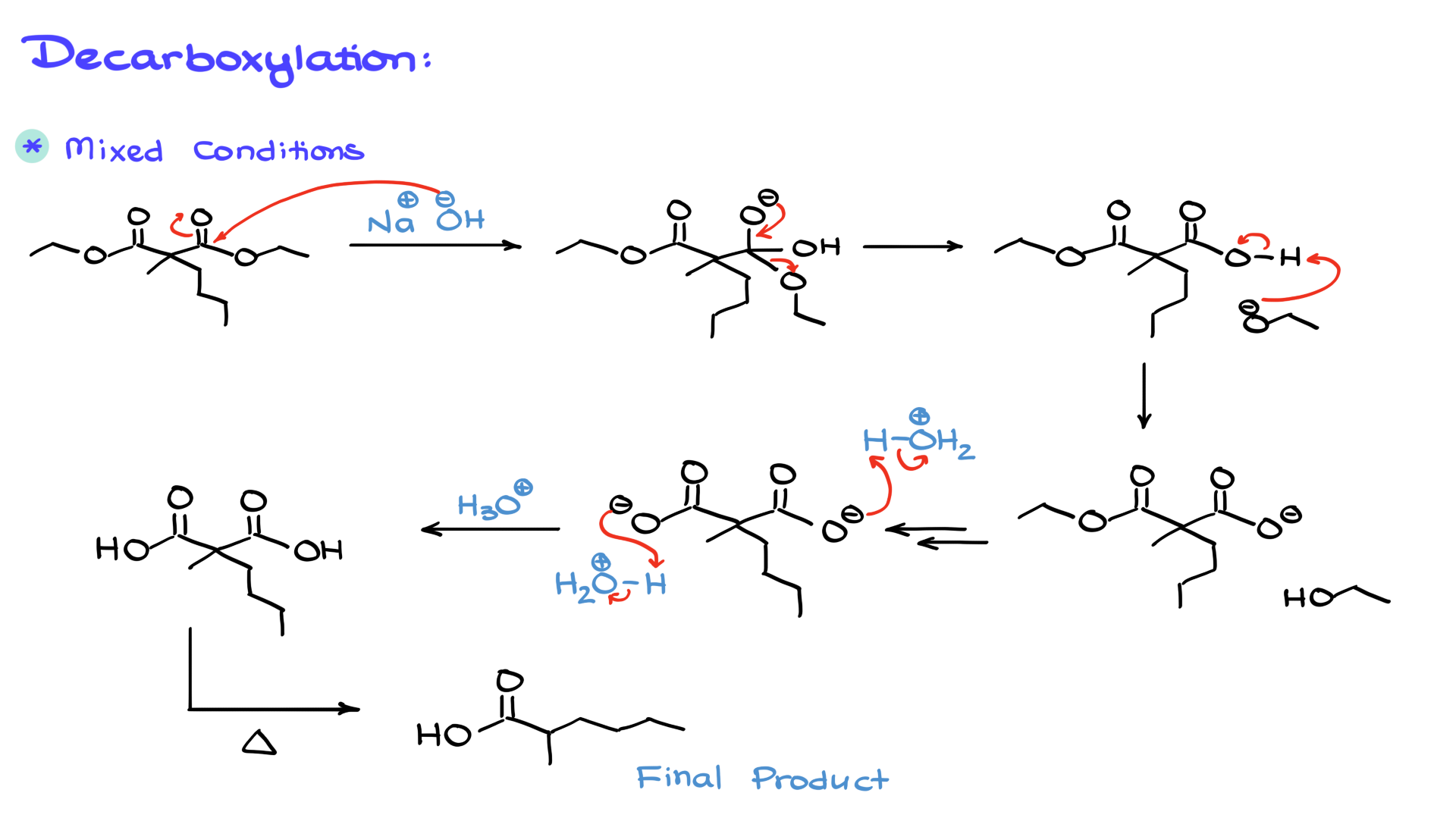
Once we have the dicarboxylic acid, we proceed to decarboxylation, which involves heating the compound to remove one of the carboxyl groups as CO₂. This occurs through a cyclic transition state, leading to an enol intermediate, which quickly tautomerizes to the final carboxylic acid product.
Summary of the Malonic Ester Synthesis
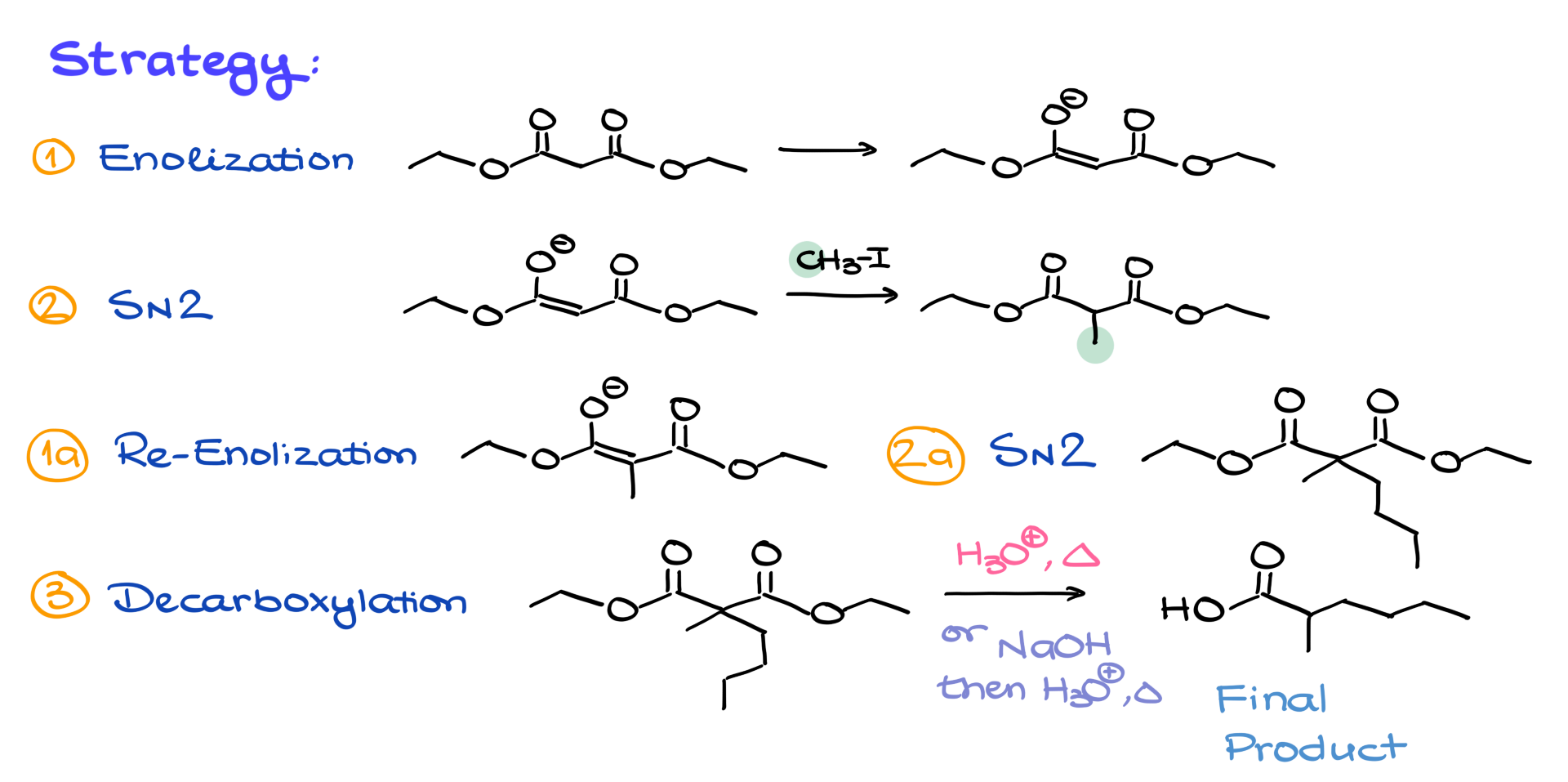
To summarize, the key steps in the Malonic Ester Synthesis are:
1. Enolization: Deprotonate the malonic ester using a base to form the enolate.
2. Alkylation: React the enolate with an alkyl halide via SN2 substitution to introduce an alkyl group.
3. (Optional) Repeat steps 1 and 2 to add a second alkyl group.
4. Hydrolysis: Convert the ester groups into carboxylic acids using either acidic or basic conditions.
5. Decarboxylation: Remove one carboxyl group through heating to yield the final carboxylic acid product.
Although this process might seem complex at first, once you practice it a few times, you’ll find that it follows a logical and predictable pattern. By carefully following each step, predicting the final product of a Malonic Ester Synthesis sequence becomes quite manageable.
Examples
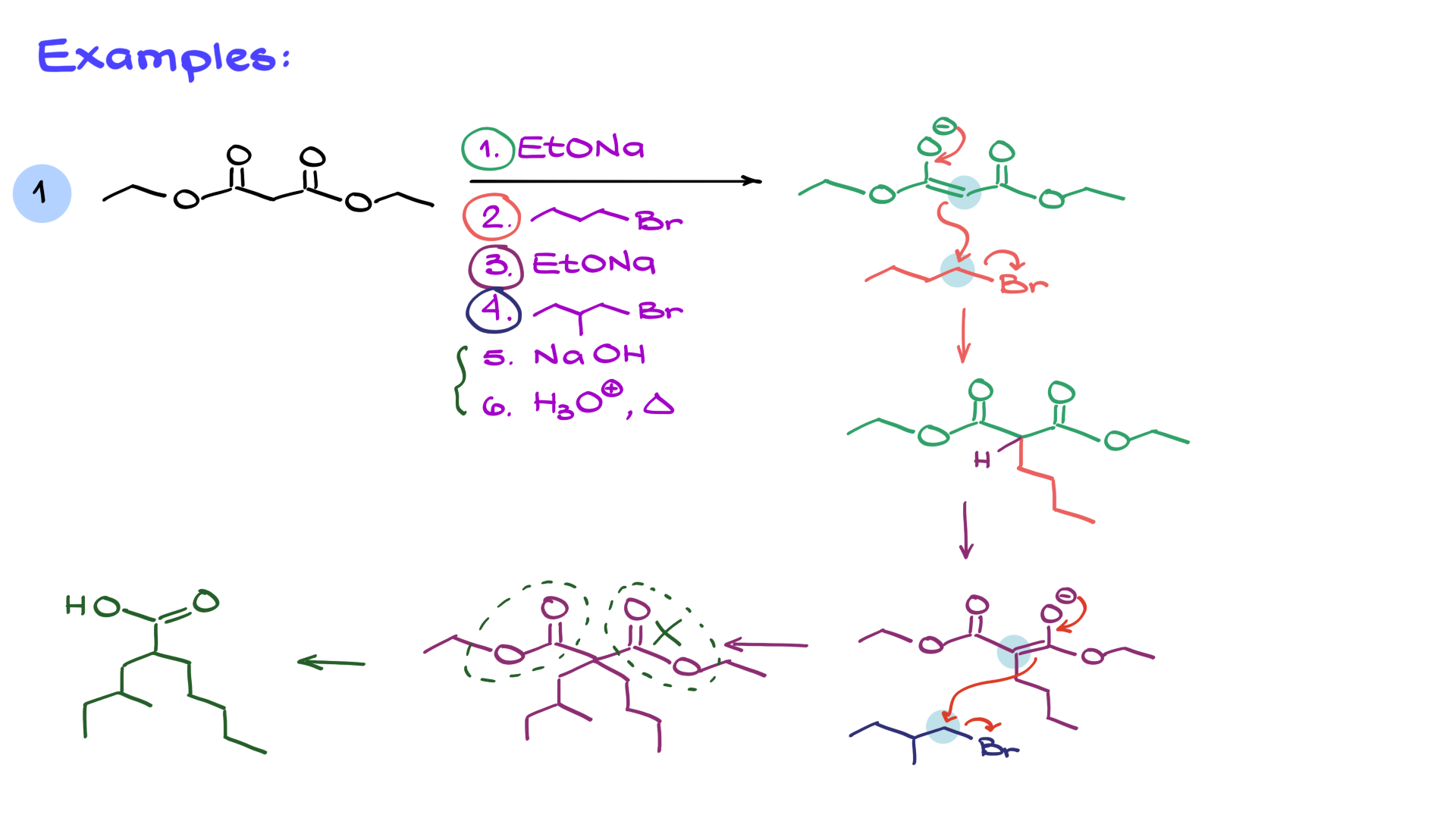
Let’s apply this to a specific case. Suppose we start with malonic ester and carry out the following sequence:
1. Treat with sodium ethoxide → forms the enolate.
2. React with butyl bromide → adds a butyl group.
3. Repeat enolization with sodium ethoxide → forms a new enolate.
4. React with another alkyl halide → adds another group.
5. Hydrolyze and decarboxylate → yields the final carboxylic acid.
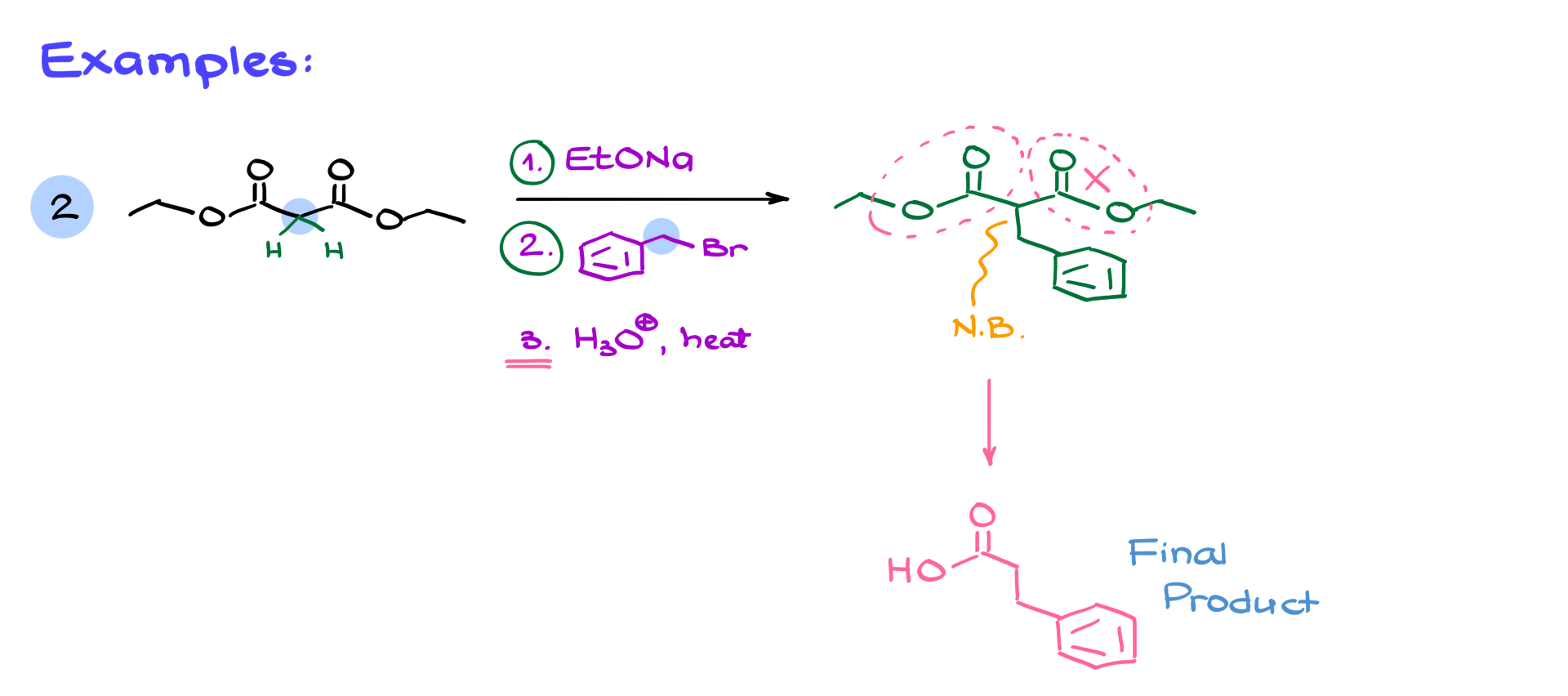
Following through the steps systematically ensures that we arrive at the correct product.
Final Thoughts
The Malonic Ester Synthesis is a powerful tool for constructing carboxylic acids with customized alkyl substituents. By mastering each step and understanding the underlying principles, you can confidently apply this method to a variety of synthetic challenges. The key is practice—once you go through a few problems, you’ll see that this reaction is both predictable and versatile.
Practice Questions
Would you like to see the answers and check your work? Become a member today or login if you’re already a member and unlock all members-only content!