Intramolecular Aldol Condensation
As any organic chemist will tell you, I love organic molecules. And we all know the universal truth: if you love organic structures, you’ve got to put a ring on it. That’s exactly what I’ll be doing today as we dive into the intramolecular aldol condensation—a reaction where we start with a dicarbonyl structure and end up with a cyclic alpha-beta unsaturated ketone, like the ones I have in the examples below. While aldol condensations can occur under both acidic and basic conditions, we typically see them in basic conditions, and that’s the chemistry I’ll be focusing on today through all my examples.
Example 1
So, let’s jump right into the first reaction. In Example 1, I have a completely symmetrical diketone, which means it doesn’t matter which side I enolize. But even though the molecule is symmetrical, each side has two enolizable positions. Let’s call them the purple enolizable position and the green enolizable position. The trick with intramolecular aldol condensations is that not every enolate will give you a desirable product. You need to consider the size of the ring you’re forming. If I enolize the green position, the resulting enolate will attack the carbonyl, forming a four-membered ring. And four-membered rings? Pretty unstable. Not the route we want to take. But if I choose the purple position, that enolate will attack the carbonyl and form a stable six-membered ring. Since four-membered rings are a no-go and six-membered rings are stable, we’ll stick with the purple enolizable position.
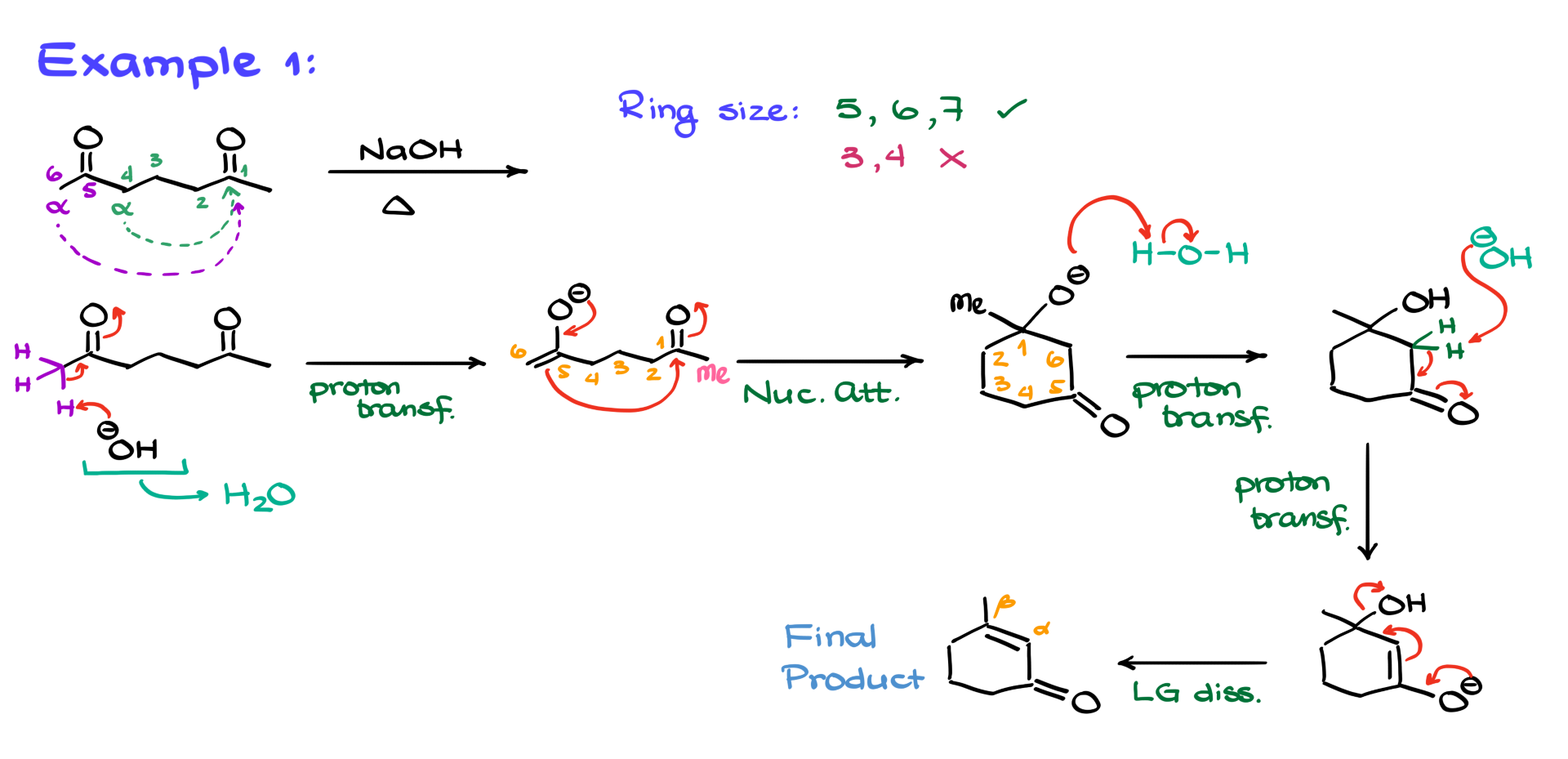
Doing this kind of pre-analysis of your molecule before jumping into the mechanism helps you quickly see which enolizable position is likely to react, saving you from brainstorming all possible outcomes, which could be overwhelming. Remember, in cyclization reactions like intramolecular aldol condensations or Dieckmann condensations, we generally favor five-, six-, or seven-membered rings. Three- or four-membered rings just don’t cut it.
Back to our reaction. I’ll redraw the molecule and highlight the purple enolizable position, showing the protons there. With the addition of a base, the first step is enolization, giving us the enolate intermediate. Now, the nucleophilic part of the molecule—the enolate—will react with the electrophilic part, which is the original carbonyl. Drawing the curved arrows from the nucleophile to the electrophile forms our six-membered ring. Drawing cyclic intermediates can be tricky, so I use the method I teach in my cyclization videos: start with a stem, draw a six-membered ring, and number it. I numbered it counterclockwise, but clockwise works too. The numbering is just an anchor to keep track of everything.
Looking at the molecule, carbon 1 has a negatively charged oxygen and a methyl group, which I’ll add in pink as Me. We’ve formed a new bond between carbons 1 and 6. Carbon 5 holds the carbonyl from our enolate, completing our intermediate. Next, we protonate this intermediate to form a neutral aldol. Working in sodium hydroxide means our solvent is likely water or something aqueous, so water acts as our proton source. In basic conditions, the most acidic species is the conjugate acid of the base we’re using, so don’t write H3O+ or H+. That’s impossible here. Acidic conditions would be a different story with a different mechanism, but in basic conditions, water is our proton donor.
Proton transfer occurs as the oxygen grabs a hydrogen from water, forming the aldol and regenerating the hydroxide ion. From here, we target the alpha position, removing protons to form the enolate again. This enolate eliminates the leaving group, producing our final product: an alpha-beta unsaturated cyclic ketone. The same final product would form under acidic conditions, though the mechanism would differ. As a challenge, try writing the mechanism under acidic conditions and see if you reach the same product.
Example 2
Moving to Example 2, we no longer have a symmetrical molecule, which means more choices and an extensive analysis to determine which position to enolize. Let’s label the enolizable positions as 1, 2, 3, and 4. Enolization at position 1 would create an awkward reach across the molecule, making a stable cycle unlikely. So, we discard that option. Position 2 offers a promising six-membered ring, as does position 3. Position 4, however, would give us an eight-membered ring, which is too large and uncommon in these reactions. So, our analysis narrows to positions 2 and 3.
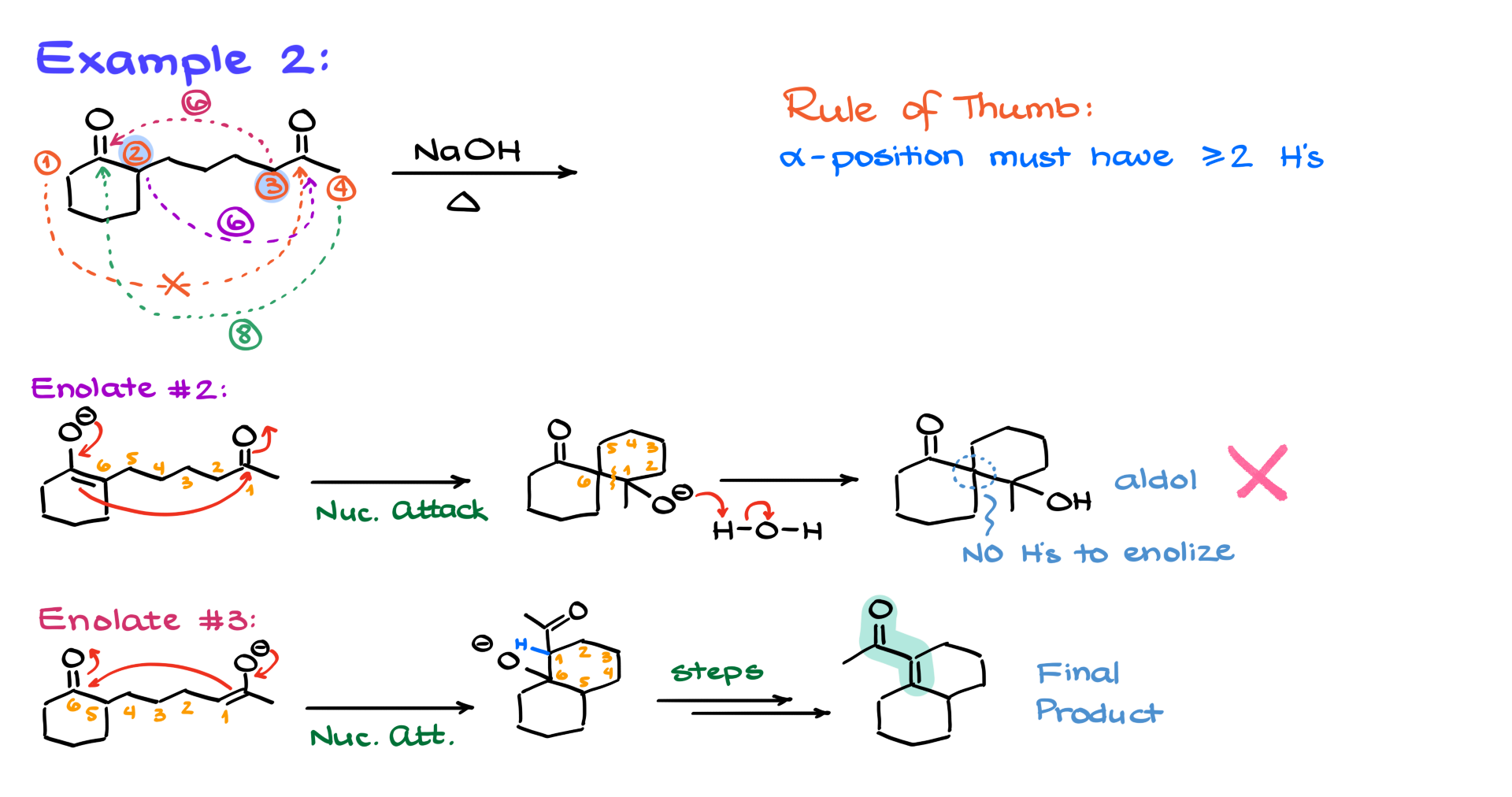
Starting with enolate 2, the nucleophilic attack on the carbonyl forms a spirocyclic compound. Numbering the atoms 1 through 6, we see a new bond between carbons 1 and 6. After protonation, we get the corresponding aldol, but here’s the problem: the alpha position lacks protons. Without those protons, we can’t form the double bond between the alpha and beta positions, halting the reaction. This is a dead end. Remember, for aldol condensations, the alpha position must have at least two hydrogens. One hydrogen isn’t enough for the elimination step. Tricks are useful only when you understand them, and now you know why two hydrogens are necessary.
Analyzing enolate 3 gives us a structure where the nucleophilic attack forms a new bond between carbons 1 and 6. This intermediate might look crowded, but we have our numbered atoms and, importantly, a proton at the alpha position. With all steps complete, we get our final product. So, while ring size matters, the enolizable position and its available protons are equally crucial. Without them, the mechanism halts, and the aldol decomposes back into starting materials, attempting cyclization from another direction. The alpha-beta unsaturated compound is thermodynamically stable due to conjugation, driving the reaction to completion.
Example 3
Now, let’s add a bit more complexity. In this next example, we again have four enolizable positions, but they’re not all the same. Positions 1, 3, and 4 are typical carbonyls with an alpha position pKa around 19. Position 2, however, sits between two carbonyls, giving it a pKa of about 9. That’s significantly more acidic, meaning position 2 is the only one deprotonated. Despite its tricky appearance, this example leaves us with only one viable option: position 2.
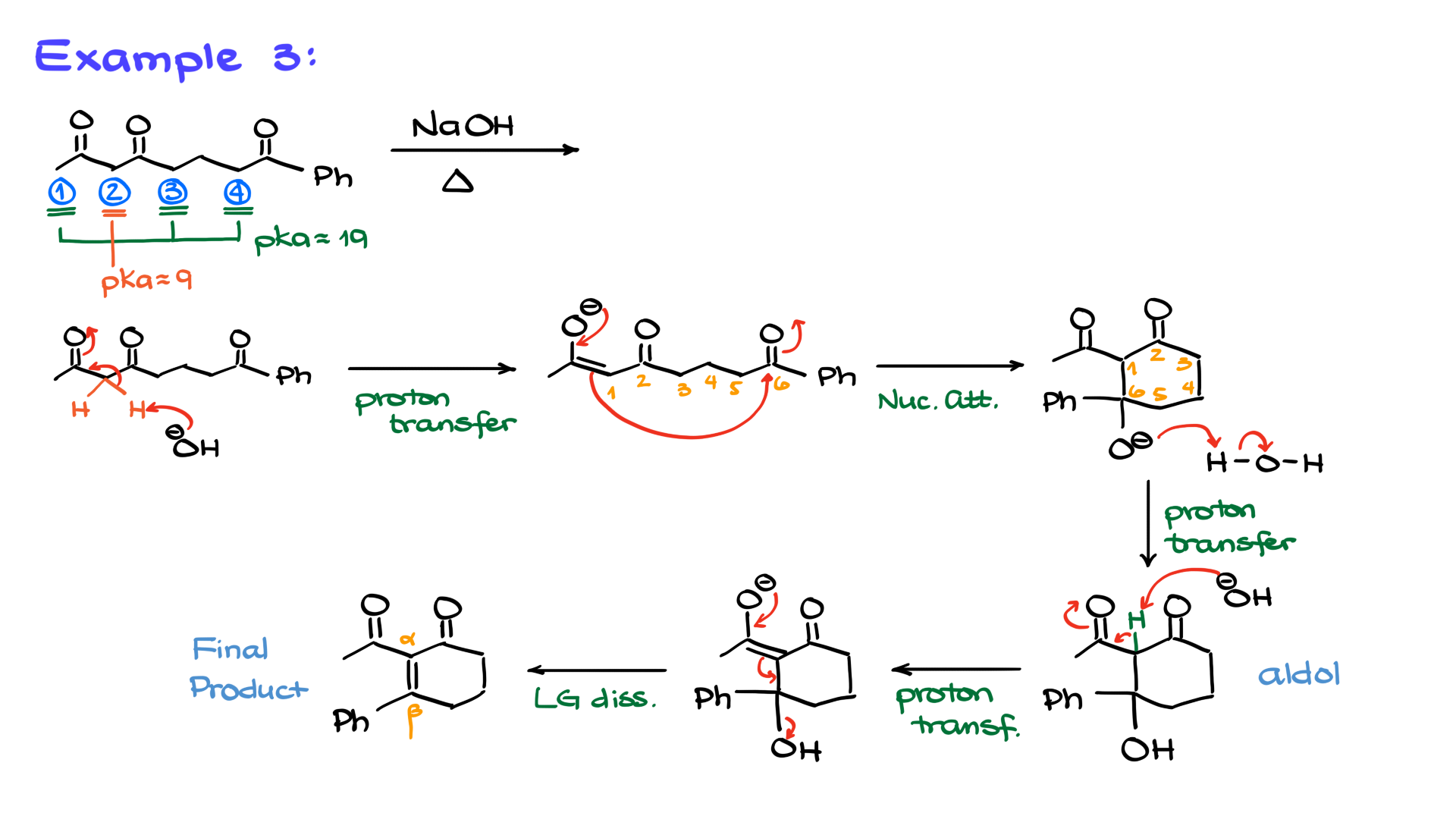
Redrawing the molecule, showing the two protons, and adding base leads to the enolate. The nucleophilic attack on the carbonyl next to the aromatic ring forms a six-membered ring. After protonation, we get our aldol, which then re-enolizes to form the final alpha-beta unsaturated cyclic compound.
Example 4
Ready for one more example?
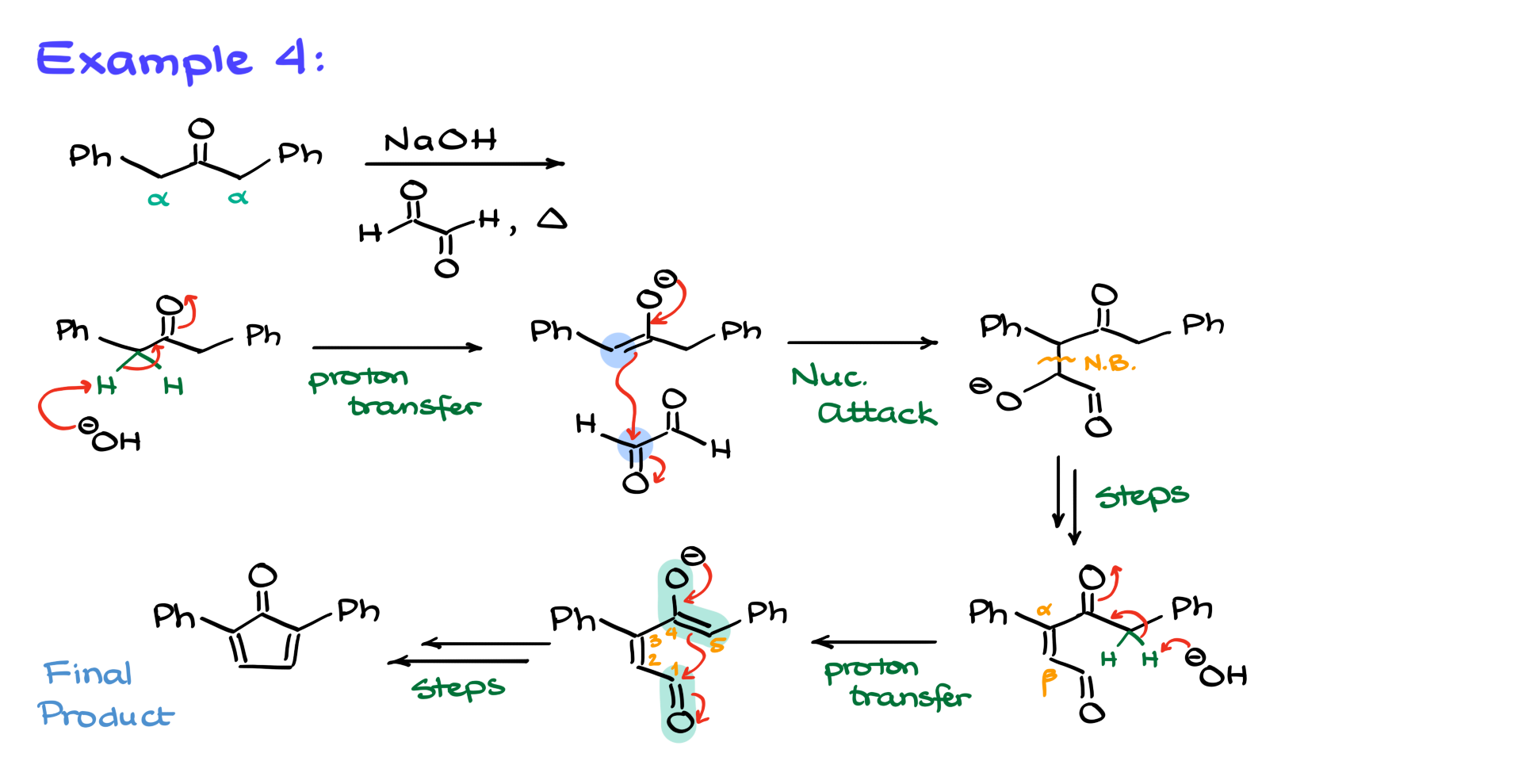
This one might seem puzzling because our starting material isn’t a dicarbonyl. It looks more like a mixed aldol condensation between a ketone and an aldehyde. But trust me, it belongs in this tutorial. The only enolizable position is in the ketone. Since the molecule is symmetrical, it doesn’t matter which alpha position we choose. Enolization, nucleophilic attack on the aldehyde, and subsequent protonation give us the aldol product with a new alpha-beta double bond. But we’re still in basic conditions, and nothing stops further enolization. This creates an enolate that can attack another carbonyl within the same molecule, leading to an intramolecular aldol condensation and a more complex final product.
This example shows how one mechanism can cascade into another, like dominoes falling until the final product is reached. As long as reaction conditions persist, the reaction continues. So, when tackling complex mechanisms, always ask yourself if the reaction is truly complete or if another step is waiting to happen—especially if your instructor loves tricky mechanisms. You never know when the reaction might keep going, forming more intricate molecules at the end.