Baeyer-Villiger Oxidation
In this tutorial, I want to talk about the Baeyer-Villiger oxidation, which is an extremely useful way of converting ketones into their corresponding esters. The Baeyer-Villiger oxidation was first reported in 1899 by Adolf von Baeyer, who later won the Nobel Prize in 1905, and his student Victor Villiger.
Overview of the Baeyer-Villiger Oxidation
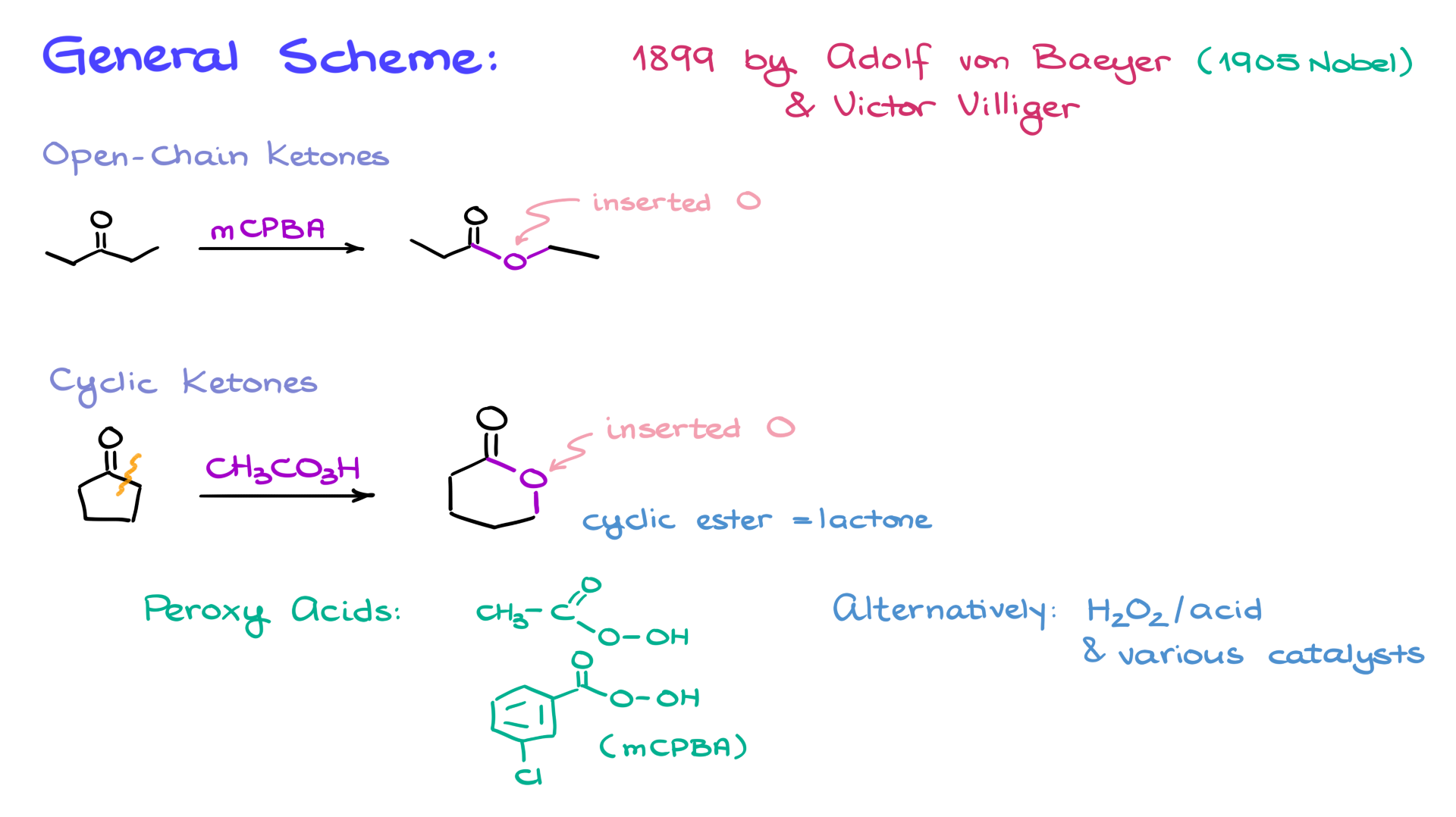
At its core, the Baeyer-Villiger oxidation takes a ketone, reacts it with a peroxy acid—such as mCPBA—and inserts an oxygen atom between the carbonyl group and an adjacent alkyl group in the molecule. This reaction works for both open-chain and cyclic ketones. For cyclic ketones, the reaction inserts an oxygen atom between the carbonyl group and the alkyl group, forming cyclic esters known as lactones.
Reactants and Conditions
As mentioned, the reaction requires a peroxy acid, such as MCPBA or peroxyacetic acid. These are the most common within the scope of organic chemistry courses, but virtually any organic peroxy acid can facilitate the reaction. Inorganic peroxy acids, like peroxysulfuric acid, can also work but are less commonly encountered in undergraduate chemistry.
Alternatively, variations of the Baeyer-Villiger oxidation use hydrogen peroxide under acidic conditions with various catalysts.
The Mechanism
Let’s jump into the mechanism, where the fun begins. We’ll start with a simple example: acetone reacting with peroxyacetic acid.
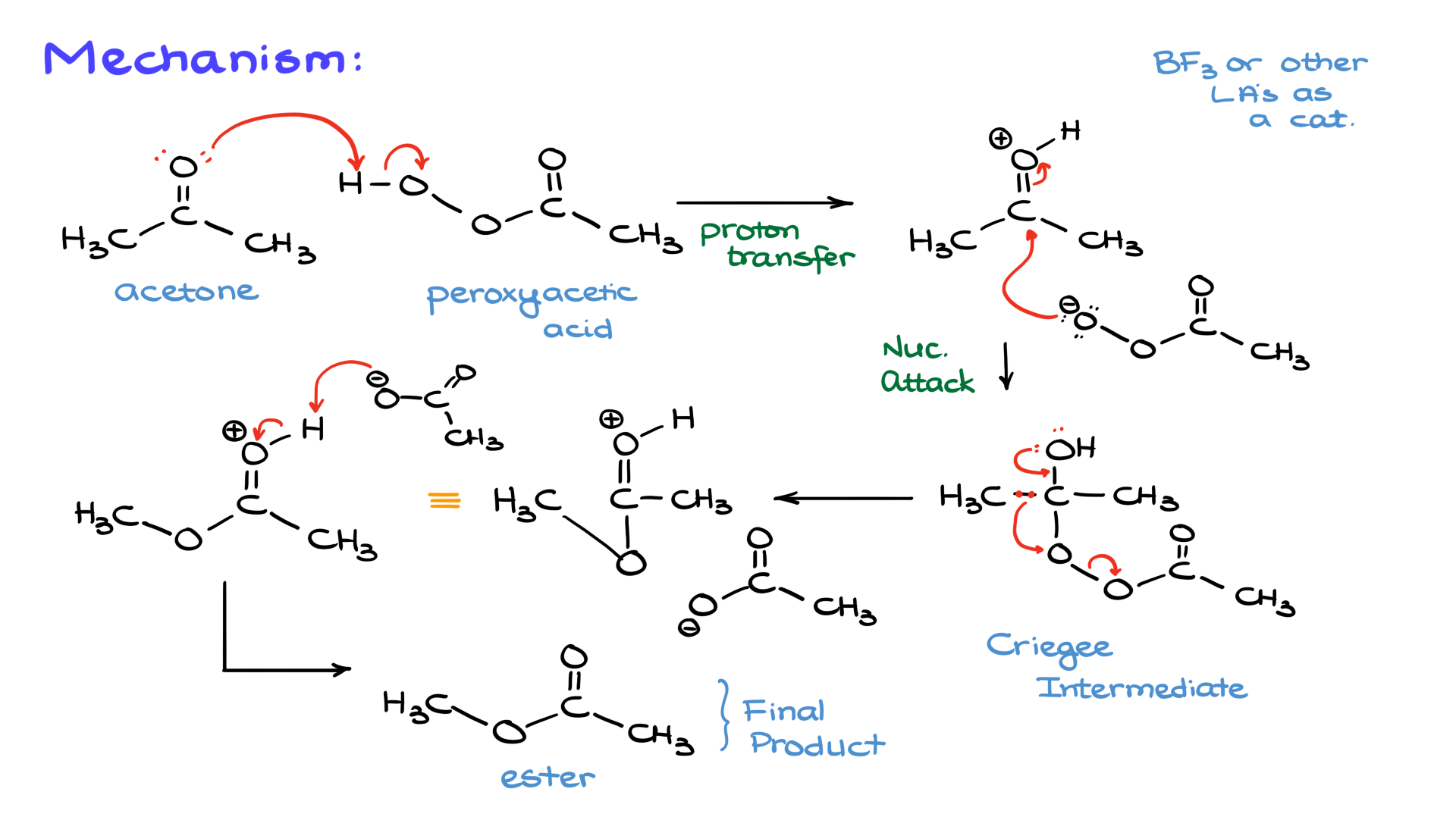
1. Protonation of the Carbonyl
The reaction begins with protonation of the carbonyl group by the peroxy acid, creating a protonated carbonyl and the conjugate base of the peroxy acid. Peroxy acids aren’t particularly strong acids, so this step doesn’t have a very high equilibrium constant. Some variations use Lewis acid catalysts like boron trifluoride to enhance the electrophilicity of the carbonyl, but the classic version of the reaction doesn’t rely on external catalysts.
2. Nucleophilic Attack
The nucleophile (from the peroxy acid) attacks the electrophilic carbonyl carbon, forming a tetrahedral intermediate. This intermediate is also called the Criegee intermediate.
3. Migration and Bond Rearrangement
The intermediate is unstable. While it can revert to the starting materials, the productive pathway involves a group migration. The electrons from the oxygen cascade downward, causing the methyl group to migrate to the oxygen atom, breaking the O-O bond. This step forms a new carbon-oxygen double bond and a single carbon-oxygen bond while cleaving the original oxygen-oxygen bond.
4. Deprotonation
The resulting protonated ester is deprotonated by the conjugate base (acetate), yielding the final ester product and acetic acid as a byproduct.
Predicting the Major Product in the Baeyer-Villiger Oxidation
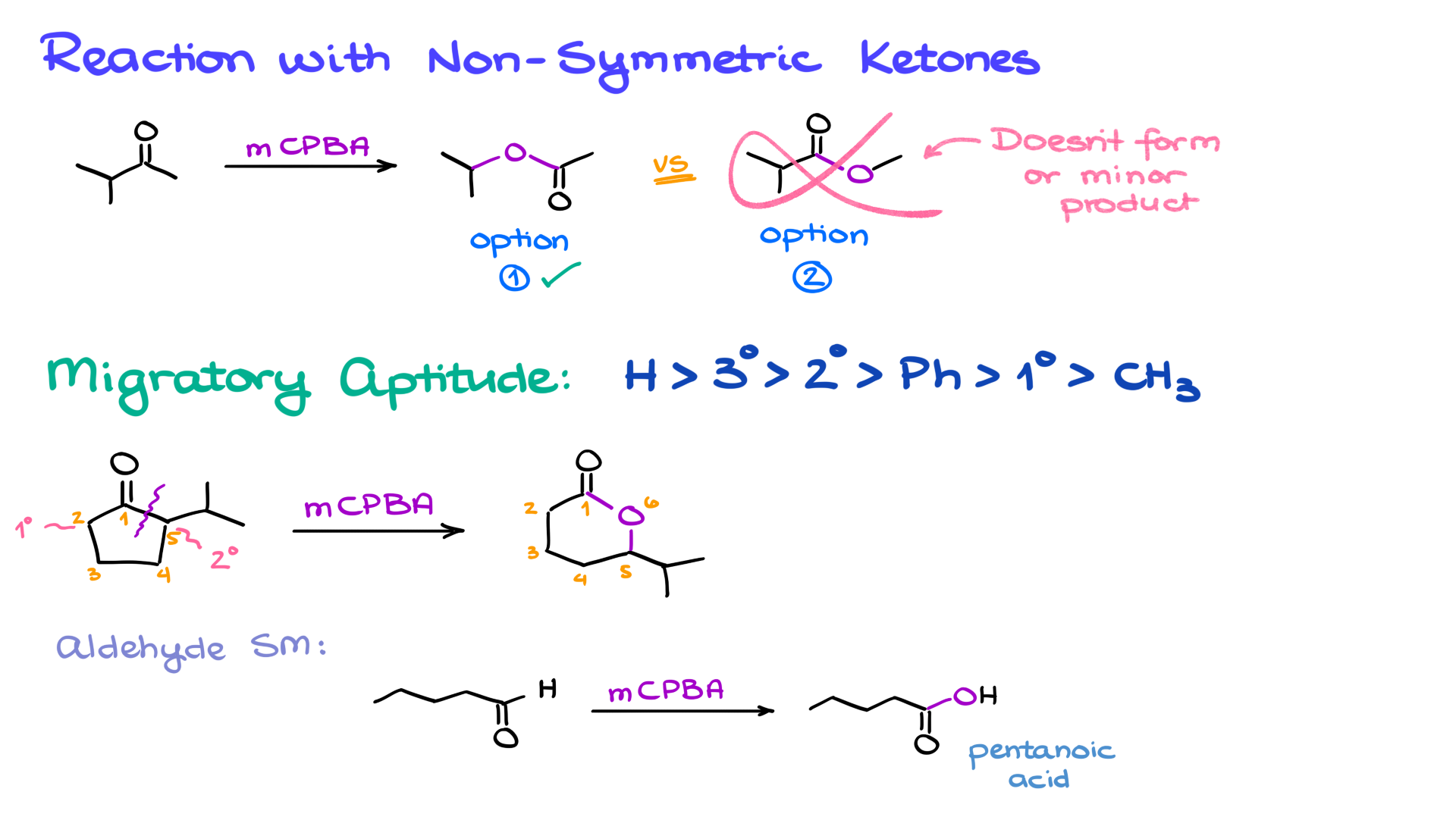
For non-symmetric ketones, such as one with an isopropyl group on one side and a methyl group on the other, the reaction typically inserts oxygen between the carbonyl and the larger group (in this case, the isopropyl group). This selectivity is due to the “migratory aptitude” of the groups, which follows this order:
H > tertiary alkyl > secondary alkyl > phenyl > primary alkyl > methyl.
This principle also applies to cyclic ketones. For example, in a five-membered ring ketone, the oxygen inserts between the carbonyl and the more substituted carbon, forming a six-membered lactone.
Application to Aldehydes
If the starting material is an aldehyde rather than a ketone, the hydrogen attached to the carbonyl migrates due to its high migratory aptitude. This leads to the formation of a carboxylic acid instead of an ester.
Stereochemistry of the Baeyer-Villiger Oxidation
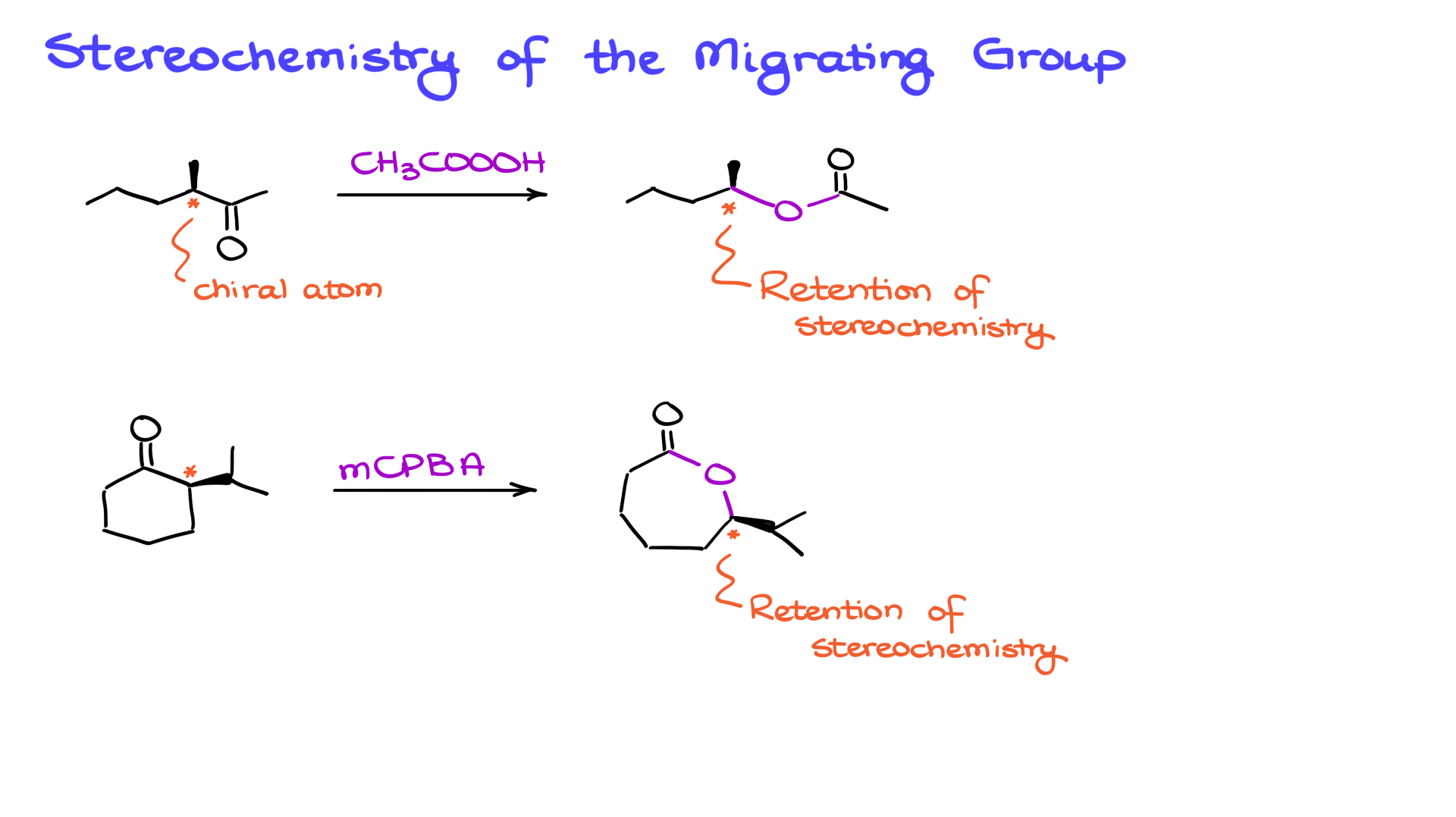
The Baeyer-Villiger oxidation retains the stereochemical configuration of chiral centers adjacent to the carbonyl. For example, if the starting material has a chiral center, the stereochemistry remains unchanged in the product. This applies to both acyclic and cyclic ketones.
Examples
In cases where mCPBA is used, it’s important to remember that mCPBA can also perform epoxidation on alkenes. However, the Baeyer-Villiger reaction generally proceeds faster than epoxidation when both functional groups are present, so the ketone is oxidized preferentially.
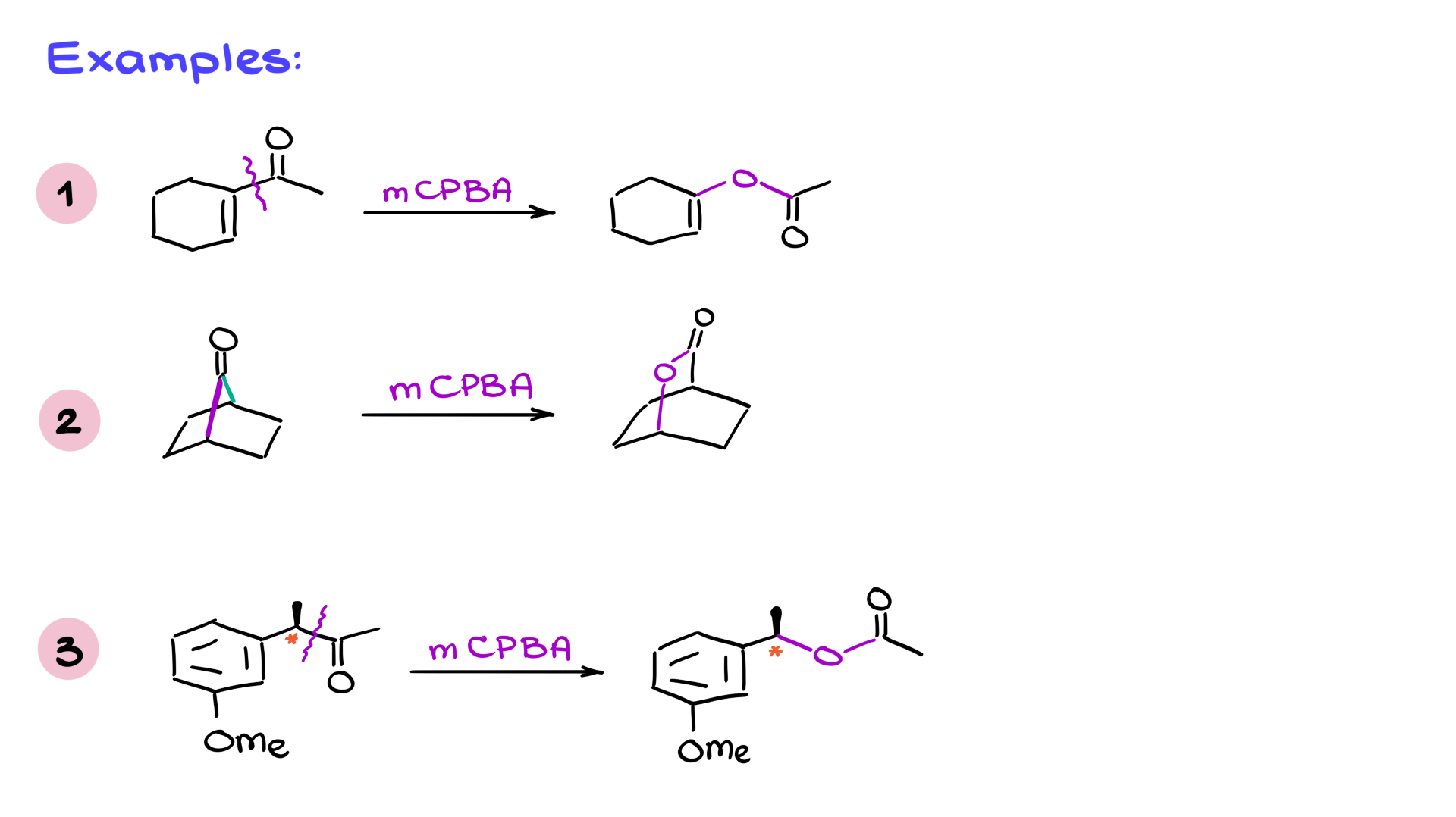
Practice Questions
Would you like to see the answers and check your work? Become a member today or login if you’re already a member and unlock all members-only content!